To put forth solar fuels as a mainstream energy production industry requires addressing the challenges that solar fuels currently face. The main challenge facing the success of solar fuels is the catalysis of the pertinent fuel-forming reactions.
Innovative Catalytic Approaches to Solar Fuel Production
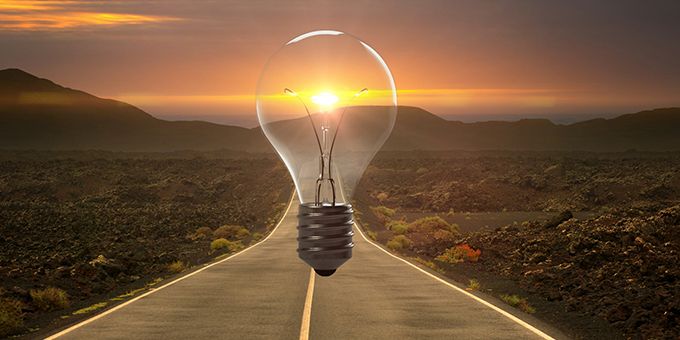
Dr. Raj Shah , Mr. Nabill Huq | Koehler Instrument Company
There is a need to address the consequences of global energy production for the preservation of our climate. The recent historical trend of climatic conditions shows the negative impacts of increased carbon footprint and greenhouse gas emissions that come with energy sources that are typical of our time. Fossil fuels like coal, petroleum, and natural gas currently dominate as the global source of energy and continue to disproportionately affect the environment and ecosystems alike. The push to transition the reliance of our energy sources to renewable and sustainable sources has been a global effort, which has become an urgent matter for the consideration of our future. As researchers continue to explore a multitude of possible renewable sources, one area of recent innovation concerns the implementation of solar fuels. Innovation in this sector is pertinent to the goal of having a sustainable energy production process in the future, as solar fuel-based energy shows the most promise for long-term and large-scale storage of renewable energy [1].
The idea of harvesting energy from the sun is not new. The solar energy the Earth receives daily makes the sun the largest renewable-energy source available to humanity [1]. Currently, the most common technology used to convert solar energy into electricity is photovoltaic solar cells [1]. These solar cells have garnered much success: new installations have increased by 40% yearly for more than a decade while dropping in price by about 80% in the last few years [1,2]. Despite the maturation of this market, there is a fundamental issue regarding the use of photovoltaic solar cells. The intermittency of solar irradiation creates large variances in the amount of energy produced, which in combination with the limitations of electrical grids, will make it difficult to store large amounts of power and to store it for extended periods [1]. This fundamental problem has researchers turning towards solar fuels as the key to a sustainable energy storage system. Solar fuels are produced from abundant resources like water and carbon dioxide, using only the sun’s energy as input [3]. These liquid fuels can be easily stored and transported for later use in producing electricity. One approach to solar fuel production is through artificial photosynthesis. Akin to natural photosynthesis, water and carbon dioxide converts to high-energy compounds with the help of sunlight [1]. The difference is that while plants use photosynthesis to create glucose for energy at a low efficiency (~1% of solar energy converted to biomass), artificial photosynthesis has a host of fuels it can produce, such as hydrogen fuel cells and alcohols with theoretical efficiencies of over 40% [4,5]. With further development of solar fuels, the implications for sustainable energy production are remarkable.
To put forth solar fuels as a mainstream energy production industry requires addressing the challenges that solar fuels currently face. The main challenge facing the success of solar fuels is the catalysis of the pertinent fuel-forming reactions. Thus, the chief source of innovation in solar fuels lies in the chemistry that drives the reaction. The difficulty with catalysis is that adding more catalyst to the reaction does not increase the rate of fuel production when using artificial devices [6]. It is the nature of the catalyst itself and how it interacts with the corresponding light-absorbing materials in the light conversion process that mainly affects reaction efficiency [6]. The main objective is to develop a catalyst that can tune the fuel-forming chemical reactions with low-energy barriers for the prospect of maximizing the efficiency of high-energy conversion for the overall production process [1]. Therefore, the development of the catalyst itself is crucial to the efficiency of the whole process.
The innovation of catalysis and the type of catalyst used depends on the fuel that is being produced. There are many options regarding fuel types that can derive its energy source from the sun. Hydrocarbons like methane and propane represent one important fuel type that can be produced using sunlight. Methane in particular has several logistical advantages for use as a fuel. Methane is the primary component in natural gas, a commonly used energy source today. Furthermore, the infrastructure to distribute, store, and utilize methane is already prevalent [7]. When methane is burned for as a source of energy, it breaks into carbon dioxide and water, which has negative ramifications on the environment. This process gets reversed when methane is produced as a solar fuel, as carbon dioxide and water become the feedstock instead, along with sunlight [8]. The current difficulty of methane production as a solar fuel include the high energy required for the arduous 8-electron/proton coupling transfer that is both kinetically and thermodynamically unfavorable [9,10,11]. Catalysts can help with the energy barrier of entry for these reactions and are the main focus of innovation for methane production as a solar fuel.
A recent development in methane production is a catalytic approach to solar fuel using a copper- and iron-based electrocatalyst. The binary CuFe electrocatalyst is used for the selective reduction of carbon dioxide to methane [12]. The copper and iron in the electrocatalyst work in union to induce an alteration to the O-C-O angle to 126.05° from its original linear structure to stimulate strong interaction with carbon dioxide and lowers the energy barrier for reaction, which helps expedite methane synthesis [12]. To create the CuFe electrocatalyst, the researchers began with growing arrays of gallium nitride nanowires on top of a silicon wafer, which serves to absorb light [12]. Through electrodeposition, particles from a copper and iron mix of 5- to 10-nanometers wide were added by using the nanowires as support [12]. This electrocatalyst, in conjunction with carbon dioxide, water, and light, can convert 51% of the light energy into methane [12]. The higher performance of the CuFe catalyst is due to the fact that this electrocatalyst offers more active centers that promote the kinetics of the process [7]. A notable advantage of this catalytic approach is that all the components needed for the setup are inexpensive, plentiful, and are commonly used in industry [8]. Despite the initial promise this system shows, there is still room for improvement. Future investigations can work on improving the efficiency and rate of methane production to make this technique practical for commercial production.
Figure 1: Spatial decoupling of the CO2 reduction reaction from light absorption and separation of charge carriers over the CuFe catalyst on the GaN nanowires [12]
A different novel method used to produce hydrocarbons like propane and methane employs the use of gold nanoparticles in combination with an ionic fluid to create a photocatalytic system that converts carbon dioxide into hydrocarbon fuel. Inspired by chlorophyll, which acts as a catalyst in natural photosynthesis, the gold nanoparticles have a strong localized surface plasmon resonance (LSPR) band hovering around 520 nm, which allows strong absorption of green light [13]. The gold nanoparticles, with a diameter of ~12 nm and a pseudospherical shape, are known to activate carbon dioxide, showing chemical stability against bulk oxidation and photocorrosion [13,14,15]. These nanoparticles are paired with an ionic liquid medium of 1-ethyl-3-methylimidazolium tetrafluoroborate (EMIM-BF4), which enhances reactivity and puts the entire photocatalyst scheme together [13]. The best performance of EMIM-BF4 for favoring C2+ production was at 5 mol% [13]. The photocatalyst works by having EMIM-BF4 promote electron transfer at the interface of the photoexcited gold nanoparticles and adsorbed carbon dioxide [13]. The ionic liquid is also used to stabilize the charged intermediates formed at the nanoparticle/solution interface that is useful for the activation of CO2, which helps facilitate multi-step reduction and C-C coupling [13]. This breakthrough in the lab still requires further investigation before it can become economically feasible. Areas of optimizations include increasing hydrocarbon production yields and refinement of chemical conversions driven by the gold nanoparticles [16]. Another future investigation includes the possible implementation of this hydrocarbon production approach on a large-scale operation.
Another fuel that is being closely investigated for its viability as a solar fuel is formic acid. Formic acid has the benefits of being a liquid at room temperature, having a high volumetric capacity as a hydrogen energy carrier (53 g H2/L), and low toxicity and flammability under normal conditions [17,18,19,20,21]. The overall chemical reaction to produce formic acid combines hydrogen and carbon dioxide, and vice versa for its decomposition, making it a practical carbon-neutral process [17,18,19,20,21]. Formic acid is also a versatile fuel as it can be directly used as a source of energy or be converted into hydrogen, which is another clean solar fuel. Recent innovations have led to the creation of the world’s first formic acid-based fuel cell, with electrical efficiency at 45% [22]. The viability of formic acid as a solar fuel is closely tied with how efficiently it can be produced, which requires catalytic innovations.
A current catalytic approach developed for formic acid production as a solar fuel employs the use of a novel photocatalyst sheet established with a cobalt-based catalyst. This photocatalyst sheet converts carbon dioxide and water into formate and oxygen with possible large-scale applications for carbon dioxide utilization [23]. The photocatalyst sheet was developed by integrating lanthanum- and rhodium-doped SrTiO3 (SrTiO3:La,Rh) and molybdenum-doped BiVO4 (BiVO4:Mo) light-absorbing particles into a gold layer using a previously established particle transfer method [23,24]. The BiVO4:Mo particles that are modified by a RuO2 catalyst strengthen the power of water oxidation when under visible light irradiation [25]. SrTiO3:La,Rh was utilized for its light-absorbing properties for the carbon dioxide reduction reaction (CO2RR) with its relatively negative conduction band [26]. The photocatalyst sheet is further modified by a phosphonated Co(II) bis(terpyridine) (CotpyP), which is a molecular catalyst for CO2RR through its immersion in a methanolic solution [23]. The selection of CotpyP as the cocatalyst was made due to its simple preparation and the ease in which it immobilizes on porous metal oxide scaffolds through its phosphonate-anchoring groups [27]. The resulting fuel generated from this system has a solar-to-formate conversion efficiency of 0.08 ± 0.01% with a formate selectivity of 97 3% with water as the electron donor [23]. Another advantage of this novel process is high stability throughout the photosynthetic reaction without the need for sacrificial reagents [23]. Despite the prospect of a large-scale production process of formic acid using these innovative photocatalyst sheets, the lackluster efficiency of the process still needs improvements. Investigative case studies into the components used for this catalytic approach will garner new innovations to make its application more practical.
Another interesting catalytic approach to formic acid production as a solar fuel is to convert plastic waste into formic acid from exposure to sunlight driven by a vanadium-based photocatalyst. The process begins by recycling non-biodegradable plastics, like polyethylene, through cascade C—C bond cleavage [28]. The selective C—C bond cleavage is done with the help of a vanadium photocatalyst, which draws solar energy to power the cleavage process [28,29]. Typical cleavage of C—C bonds require energy-intensive high temperatures to occur but sourcing this energy from sunlight instead provides an ecological solution to the process [30]. The experimentation of the reaction to create formic acid was under the following conditions: 0.50 mL of acetonitrile (solvent), 0.10*10-3 M of substrate, 5 mol% of the vanadium-based catalyst, 0.40 * 10-3 M of 1,4-Cyclohexadiene, O2 as the terminal oxidant, and a light-emitting diode (45 W) [28]. When tested against polyethylene, full substrate conversion was observed after six days, in which some products included formic acid and alkyl formates [28]. Although the practicality of this process for solar fuel production is currently unreasonable, this is the first chemical treatment that can completely break down non-biodegradable plastic using sunlight and a photocatalyst that can produce useful products like formic acid. Further development of this process shows promise of the viability of converting plastic waste into energy-rich solar fuels.
Figure 2: Chemical structure of the vanadium photocatalyst used in formic acid production [28]
When developing solar fuels that have the possibility of becoming a prominent source of energy, the practicality of such a process is one of the biggest concerns. Thus, the idealistic solar fuel is one that is already built into the fabric of our current infrastructure. One solar fuel that fits this criterion is ethanol. Ethanol is a widely used chemical in many industries like pharmaceuticals and cosmetics and is a prominent ingredient in U.S. gasoline. Pure ethanol is non-toxic, biodegradable, and ethanol-gasoline mixtures burn cleaner than pure gasoline [31]. Currently, the most common method for producing ethanol is the fermentation of the starch and sugars found in corn, sugar cane, and sugar beets [32]. This current method is problematic concerning the resources put into bioethanol production rather than for the purpose of growing food crops [31]. Ethanol shows much promise as a carbon-neutral solar fuel, as the production of ethanol reuses the carbon dioxide that is emitted from when ethanol is burned for energy [33]. With proper research and development of ethanol production from carbon dioxide and water, there is a real chance of integrating solar fuels into the future ecosystem of energy production.
An original approach to ethanol production as a solar fuel applies to a recent breakthrough of a carbon-supported copper electrocatalyst that converts carbon dioxide and water into ethanol. The electrocatalyst is synthesized from a blended Cu-Li method that results in copper atoms transferred onto a carbon surface once the lithium is leached away from a Cu-LiOH mixture [34]. In addition, the electrocatalyst comprises of single-atom (SA) copper, which has a form of uniformly dispersed copper atoms that follow a similar path in forming transition and active site intermediates under reaction conditions [34]. The faradaic efficiency, which is a measure of electrocatalytic selectivity, of carbon dioxide-to-ethanol conversion is favored by the high initial dispersion of SA copper [34]. The electrochemical reaction employs the use of an external electric field, which stimulates a reversible transformation between Cu SAs and Cun (n = 3 and 4) in which Cun ligates with the surface hydroxyl group and acts as the temporary active site to bind with CO2 in the electrolyte [34]. The catalysis loop closes when the electric field is turned off, reverting the Cun into Cu SA ions [34]. The resulting faradaic efficiency of this process is 91%, which is much higher than any previously reported process [34]. Additionally, the catalyst showed stable operation over 16 hours, maintaining a faradaic efficiency of ~90% [34]. One advantage of this novel ethanol production process is that the low temperature and pressure requirements of running the process allows it to start and stop promptly in response to the intermittent supply of electrical energy supplied from the sun [33]. With additional investigation into the underlying science of the atomically dispersed electrocatalyst, further improvements will aid in the practical application of ethanol as a solar fuel.
Figure 3: Synthesis of carbon-supported copper electrocatalyst [34]
The novel catalytic systems being developed today show a bright future for solar fuel production. The success of solar fuels will chiefly derive from the new electrocatalyst and photocatalyst systems developed from a host of different chemicals. As we continue to master catalysis, solar fuels will one day be at the forefront of renewable energy production. Whether the resulting fuel is hydrocarbon-based, formic acid, ethanol, or one of the variety of solar fuels available, these fuels make solar energy a versatile option for long-term energy storage. Implementing solar fuels into the global energy production scene will not only be an effective method of harnessing solar energy that has been long underutilized, but also for reducing the excess carbon dioxide in our climate. With liquid fuels being a dominant source for energy consumption, the solar-to-fuel conversion will play a monumental role in creating fossil fuel alternatives to storable and transportable energy [35]. Currently, a solar fuel industry does not exist. With continued investment into solar fuel technology, in particular to developing efficient catalysts, the prospect of an entire market dedicated to producing clean, renewable, and sustainable fuel can become a genuine phenomenon.
Dr. Raj Shah is a Director at Koehler Instrument Company in New York, where he has worked for the last 25 years. An expert in the filed of alternative energy, he is an elected Fellow by his peers at IChemE, STLE, AIC, NLGI, INSTMC, CMI, The Energy Institute and The Royal Society of Chemistry. A Ph.D in Chemical Engineering from the Pennsylvania state University and a Fellow from the Chartered Management Institute, London, he is also a Chartered Scientist with the Science Council, a Chartered Petroleum Engineer with the Energy Institute and a Chartered Engineer with the Engineering council, UK. An ASTM Eagle award recipient, Dr. Shah recently co-edited the bestseller, “Fuels and Lubricants handbook”, details of which are available at
https://www.astm.org/DIGITAL_LIBRARY/MNL/SOURCE_PAGES/MNL37-2ND_foreword.pdf
A 2020 recipient of the illustrious Tau Beta Pi eminent engineer title, he is currently an adjunct Professor in the department of Material Science and Chemical engineering at State University of New York, Stony Brook, NY . He has over 300 publications and volunteers on Advisory board of directors at several US universities. More information on Raj can be found at
https://www.che.psu.edu/news-archive/2018/Alumni-Spotlight-Raj-Shah.aspx
Mr. Nabill Huq is part of a thriving internship program at Koehler Instrument company and a student of chemical engineering at State University of New York, Stony Brook, where Dr. Shah currently heads the External advisory board of directors.
References:
-
Hammarström, L. (2016). Catalyst: Chemistry’s Role in Providing Clean and Affordable Energy for All. Chem, 1(4), 515-518. doi:10.1016/j.chempr.2016.09.002
-
Photovoltaics Report. (2020, September 16). Retrieved October 24, 2020, from https://www.ise.fraunhofer.de/content/dam/ise/de/documents/publications/studies/Photovoltaics-Report.pdf
-
DOE Explains...Solar Fuels. (n.d.). Retrieved October 25, 2020, from https://www.energy.gov/science/doe-explainssolar-fuels
-
Sun, L., Hammarström, L., Åkermark, B., & Styring, S. (2001). Towards artificial photosynthesis: Ruthenium–manganese chemistry for energy production. Chemical Society Reviews, 30(1), 36-49. doi:10.1039/a801490f
-
Hanna, M. C., & Nozik, A. J. (2006). Solar conversion efficiency of photovoltaic and photoelectrolysis cells with carrier multiplication absorbers. Journal of Applied Physics, 100(7), 074510. doi:10.1063/1.2356795
-
Wadsworth, B. L., Beiler, A. M., Khusnutdinova, D., Cruz, E. A., & Moore, G. F. (2019). Interplay between Light Flux, Quantum Efficiency, and Turnover Frequency in Molecular-Modified Photoelectrosynthetic Assemblies. Journal of the American Chemical Society, 141(40), 15932-15941. doi:10.1021/jacs.9b07295
-
Bongartz, D., Doré, L., Eichler, K., Grube, T., Heuser, B., Hombach, L. E., . . . Mitsos, A. (2018). Comparison of light-duty transportation fuels produced from renewable hydrogen and green carbon dioxide. Applied Energy, 231, 757-767. doi:10.1016/j.apenergy.2018.09.106
-
Service, R. (2020, January 09). Taking a cue from plants, new chemical approach converts carbon dioxide to valuable fuel. Retrieved October 25, 2020, from https://www.sciencemag.org/news/2020/01/taking-cue-plants-new-chemical-approach-converts-carbon-dioxide-valuable-fuel
-
Kuhl, K. P., Hatsukade, T., Cave, E. R., Abram, D. N., Kibsgaard, J., & Jaramillo, T. F. (2014). Electrocatalytic Conversion of Carbon Dioxide to Methane and Methanol on Transition Metal Surfaces. Journal of the American Chemical Society, 136(40), 14107-14113. doi:10.1021/ja505791r
-
Mota, F. M., & Kim, D. H. (2019). From CO2 methanation to ambitious long-chain hydrocarbons: Alternative fuels paving the path to sustainability. Chemical Society Reviews, 48(1), 205-259. doi:10.1039/c8cs00527c
-
Rao, H., Lim, C., Bonin, J., Miyake, G. M., & Robert, M. (2018). Visible-Light-Driven Conversion of CO2 to CH4 with an Organic Sensitizer and an Iron Porphyrin Catalyst. Journal of the American Chemical Society, 140(51), 17830-17834. doi:10.1021/jacs.8b09740
-
Zhou, B., Ou, P., Pant, N., Cheng, S., Vanka, S., Chu, S., . . . Mi, Z. (2020). Highly efficient binary copper−iron catalyst for photoelectrochemical carbon dioxide reduction toward methane. Proceedings of the National Academy of Sciences, 117(3), 1330-1338. doi:10.1073/pnas.1911159117
-
Yu, S., & Jain, P. K. (2019). Plasmonic photosynthesis of C1–C3 hydrocarbons from carbon dioxide assisted by an ionic liquid. Nature Communications, 10(1). doi:10.1038/s41467-019-10084-5
-
Yu, S., Wilson, A. J., Heo, J., & Jain, P. K. (2018). Plasmonic Control of Multi-Electron Transfer and C–C Coupling in Visible-Light-Driven CO2 Reduction on Au Nanoparticles. Nano Letters, 18(4), 2189-2194. doi:10.1021/acs.nanolett.7b05410
-
Chen, Y., Li, C. W., & Kanan, M. W. (2012). Aqueous CO2 Reduction at Very Low Overpotential on Oxide-Derived Au Nanoparticles. Journal of the American Chemical Society, 134(49), 19969-19972. doi:10.1021/ja309317u
-
Dockrill, P. (2019). New Artificial Photosynthesis Breakthrough Uses Gold to Turn CO2 Into Liquid Fuel. Retrieved October 25, 2020, from https://www.sciencealert.com/new-artificial-photosynthesis-breakthrough-uses-gold-to-turn-co2-into-liquid-fuel
-
Eppinger, J., & Huang, K. (2016). Formic Acid as a Hydrogen Energy Carrier. ACS Energy Letters, 2(1), 188-195. doi:10.1021/acsenergylett.6b00574
-
Mellmann, D., Sponholz, P., Junge, H., & Beller, M. (2016). Formic acid as a hydrogen storage material – development of homogeneous catalysts for selective hydrogen release. Chemical Society Reviews, 45(14), 3954-3988. doi:10.1039/c5cs00618j
-
Fukuzumi, S., & Suenobu, T. (2013). Hydrogen storage and evolution catalysed by metal hydride complexes. Dalton Trans., 42(1), 18-28. doi:10.1039/c2dt31823g
-
Fukuzumi, S. (2008). Bioinspired Energy Conversion Systems for Hydrogen Production and Storage. European Journal of Inorganic Chemistry, 2008(9), 1351-1362. doi:10.1002/ejic.200701369
-
Wang, W., Himeda, Y., Muckerman, J. T., Manbeck, G. F., & Fujita, E. (2015). CO2 Hydrogenation to Formate and Methanol as an Alternative to Photo- and Electrochemical CO2 Reduction. Chemical Reviews, 115(23), 12936-12973. doi:10.1021/acs.chemrev.5b00197
-
Papageorgiou, N. (2018, March 20). The world's first formic acid-based fuel cell. Retrieved October 25, 2020, from https://actu.epfl.ch/news/the-world-s-first-formic-acid-based-fuel-cell/
-
Wang, Q., Warnan, J., Rodríguez-Jiménez, S. et al. Molecularly engineered photocatalyst sheet for scalable solar formate production from carbon dioxide and water. Nat Energy 5, 703–710 (2020). https://doi.org/10.1038/s41560-020-0678-6
-
Minegishi, T., Nishimura, N., Kubota, J., & Domen, K. (2013). Photoelectrochemical properties of LaTiO2N electrodes prepared by particle transfer for sunlight-driven water splitting. Chemical Science, 4(3), 1120. doi:10.1039/c2sc21845c
-
Lin, F., Wang, D., Jiang, Z., Ma, Y., Li, J., Li, R., & Li, C. (2012). Photocatalytic oxidation of thiophene on BiVO4with dual co-catalysts Pt and RuO2under visible light irradiation using molecular oxygen as oxidant. Energy Environ. Sci., 5(4), 6400-6406. doi:10.1039/c1ee02880d
-
Ma, X., Wu, Z., Roberts, E. J., Han, R., Rao, G., Zhao, Z., . . . Osterloh, F. E. (2019). Surface Photovoltage Spectroscopy Observes Sub-Band-Gap Defects in Hydrothermally Synthesized SrTiO3 Nanocrystals. The Journal of Physical Chemistry C, 123(41), 25081-25090. doi:10.1021/acs.jpcc.9b06727
-
Leung, J. J., Warnan, J., Ly, K. H., Heidary, N., Nam, D. H., Kuehnel, M. F., & Reisner, E. (2019). Solar-driven reduction of aqueous CO2 with a cobalt bis(terpyridine)-based photocathode. Nature Catalysis, 2(4), 354-365. doi:10.1038/s41929-019-0254-2
-
Gazi, S., Đokić, M., Chin, K. F., Ng, P. R., & Soo, H. S. (2019). Visible Light–Driven Cascade Carbon–Carbon Bond Scission for Organic Transformations and Plastics Recycling. Advanced Science, 6(24), 1902020. doi:10.1002/advs.201902020
-
Gazi, S., Đokić, M., Moeljadi, A. M., Ganguly, R., Hirao, H., & Soo, H. S. (2017). Kinetics and DFT Studies of Photoredox Carbon–Carbon Bond Cleavage Reactions by Molecular Vanadium Catalysts under Ambient Conditions. ACS Catalysis, 7(7), 4682-4691. doi:10.1021/acscatal.7b01036
-
Lavars, N. (2019, December 12). Sunlight turns plastic waste into key element of hydrogen fuel cells. Retrieved October 25, 2020, from https://newatlas.com/materials/light-plastic-waste-hydrogen-fuel-cells/
-
U.S. Energy Information Administration - EIA - Independent Statistics and Analysis. (n.d.). Retrieved October 25, 2020, from https://www.eia.gov/energyexplained/biofuels/ethanol-and-the-environment.php
-
U.S. Energy Information Administration - EIA - Independent Statistics and Analysis. (n.d.). Retrieved October 25, 2020, from https://www.eia.gov/energyexplained/biofuels/ethanol.php
-
O'Neill, M. (2020, September 08). Breakthrough Electrocatalyst Turns Carbon Dioxide Into Ethanol. Retrieved October 25, 2020, from https://scitechdaily.com/breakthrough-electrocatalyst-turns-carbon-dioxide-into-ethanol/
-
Xu, H., Rebollar, D., He, H., Chong, L., Liu, Y., Liu, C., . . . Xu, T. (2020). Highly selective electrocatalytic CO2 reduction to ethanol by metallic clusters dynamically formed from atomically dispersed copper. Nature Energy, 5(8), 623-632. doi:10.1038/s41560-020-0666-x
-
Solar Fuels. (n.d.). Retrieved October 25, 2020, from https://www.nrel.gov/csp/solar-fuels.html
The content & opinions in this article are the author’s and do not necessarily represent the views of AltEnergyMag
Comments (0)
This post does not have any comments. Be the first to leave a comment below.
Featured Product
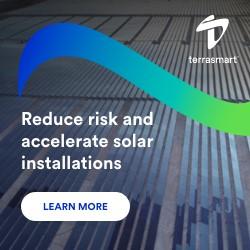